INTERFACE PHENOMENA IN COMPLEX NANO-OXIDES
Progress in nanotechnology requires new approaches to materials synthesis that make it possible to induce new functionalities down to the smallest scales. An objective of materials research is to achieve an enhanced control over the physical properties of materials such as ferromagnets, ferroelectrics and superconductors. In this context, complex oxides and inorganic perovskites are attractive because slight adjustments of the atomic structures can produce large variations in their physical macroscopic responses and result in multiple functionalities. Thin films oxides often present radically different physical properties than bulk materials, frequently due to epitaxial strain, and interface effects are paramount to understand these new properties. A particularly interesting strategy is strain engineering, which can modify the interatomic distances and even the chemistry of the film to produce novel physical phenomena. In the case of complex oxides, due to the strong correlation between chemical composition, lattice and electronic structure, modifying the growth conditions, epitaxial strain and interfase phenomena can induced multiple exciting properties such as ferromagnetism, ferroelectricity, metallicity, multiferroicity or superconductivity among others. To confront this challenge, advanced Transmission Electron Microscopy techniques provides structural and chemical information with atomic resolution to unveil the microscopic origin of this interface phenomena in complex oxides at the nanoscale.
For instance, ferroic oxides often contain ferroelastic domains. The intrinsic symmetry breaking that takes place at the domain walls can induce properties absent from the domains themselves, such as magnetic or ferroelectric order and other functionalities, as well as coupling between them. Moreover, large domain wall densities create intense strain gradients, which can also affect the material’s properties. Actually, large local stresses induces a high density of domain walls that promote the formation of new structural and chemical phases. In this case we have characterized by aberration-corrected STEM a new two-dimensional ferromagnetic phase at the domain walls of the orthorhombic perovskite terbium manganite (TbMnO3), grown in thin layers under epitaxial strain on strontium titanate (SrTiO3) substrates. A comprehensive characterization of the structure and chemical composition of this novel ferromagnetic phase by means of atomically resolved HAADF and EELS have evidenced that this new phase, yet to be created by standard chemical routes, is characterized by the selective substitution of alternate Tb atoms in the perovskite structure by Mn atoms. The density of the two-dimensional sheets can be tuned by changing the film thickness or the substrate lattice parameter (that is, the epitaxial strain), and the distance between sheets can be made as small as 5 nanometres in ultrathin films, such that the new phase at domain walls represents up to 25 per cent of the film volume. The general concept of using domain walls of epitaxial oxides to promote the formation of unusual phases may be applicable to other materials systems, thus giving access to new classes of nanoscale materials for applications in nanoelectronics and spintronics.
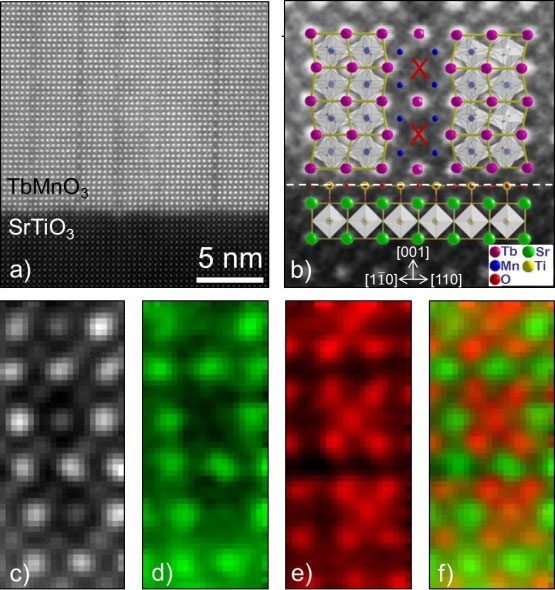
a) HAADF-STEM image of the TbMnO3–SrTiO3 interface. b) Domain wall of TbMnO3 close to the interface with the SrTiO3 substrate, with the proposed atomic model superimposed. c–f) STEM-EELS spectrum image of the domain wall; c) HAADF signal, d) Tb signal, e) Mn signal, f) Color map of Tb (green) and Mn (red).
Atomic-resolution observation of polar states in 10-nm-thick epitaxial SrMnO3 films grown by pulsed laser deposition on SrTiO3 (100). Aberration-corrected Scanning Transmission Electron Microscopy experiments have been carried out to determine the strain fields and the local atomic displacements within the unit cell through the quantitative analysis of Annular Bright Field (ABF) images with atomic resolution. ABF enables the simultaneous imaging of heavy and light atoms, which enable the visualization of the oxygen octahedra distortions in perovskite oxides as well as the off centering of the cations. As a result, we map the cell-by-cell electric polarization of SrMnO3 at atomic resolution, both far from and near the domain walls, and observe polar rotations along the growth directions resulting from vertical strain gradients [3]. This phenomena, the so-called flexoelectricity, is originated by a gradual distribution of oxygen vacancies across the film thickness and the subsequent change of the out-of-plane lattice parameter, according to electron energy loss spectroscopy. Thus, this work present a chemistry-mediated route to induce polar rotations in oxygen-deficient multiferroic films, resulting in flexoelectric polar rotations and with potentially enhanced piezoelectricity.
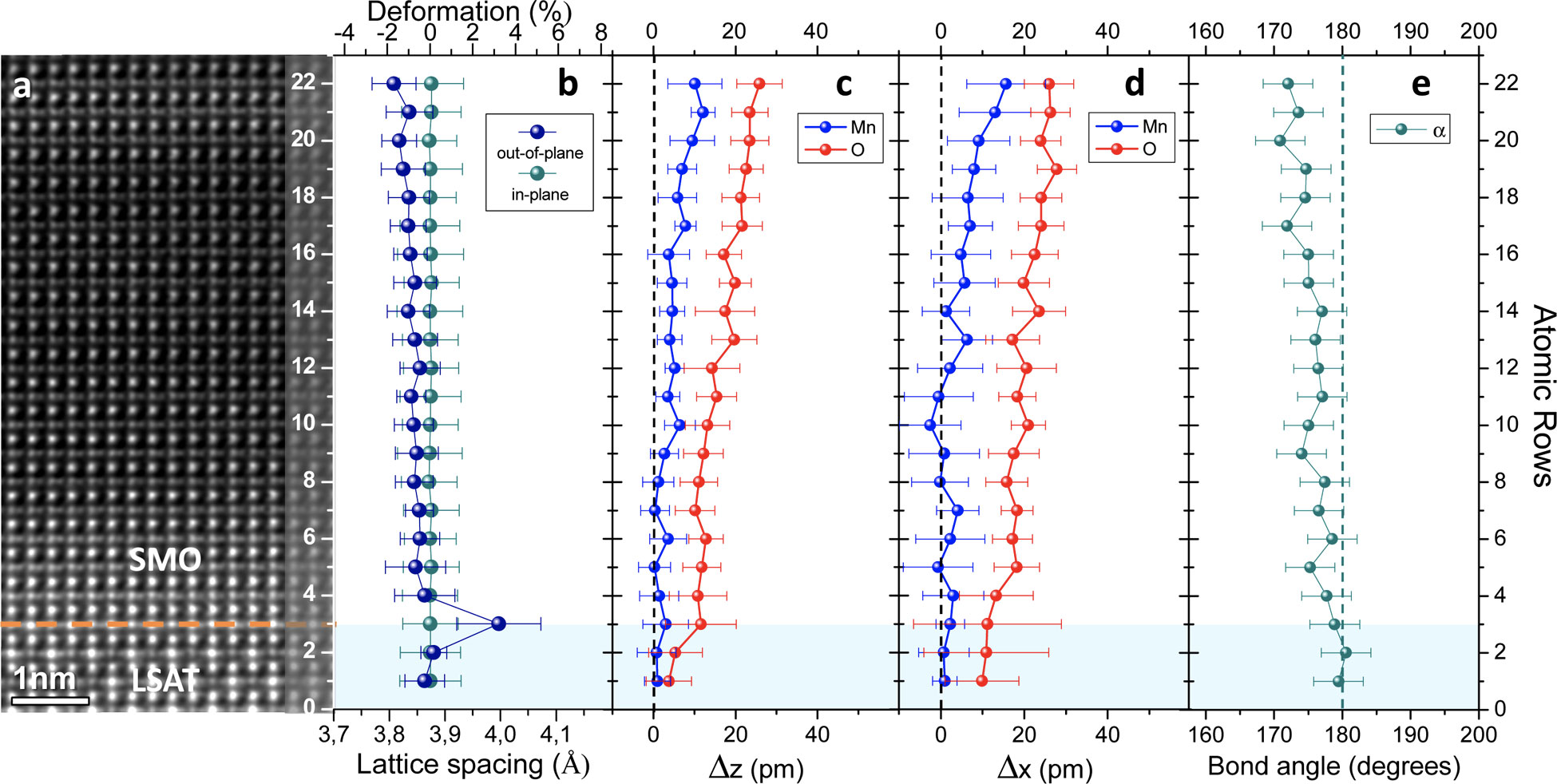
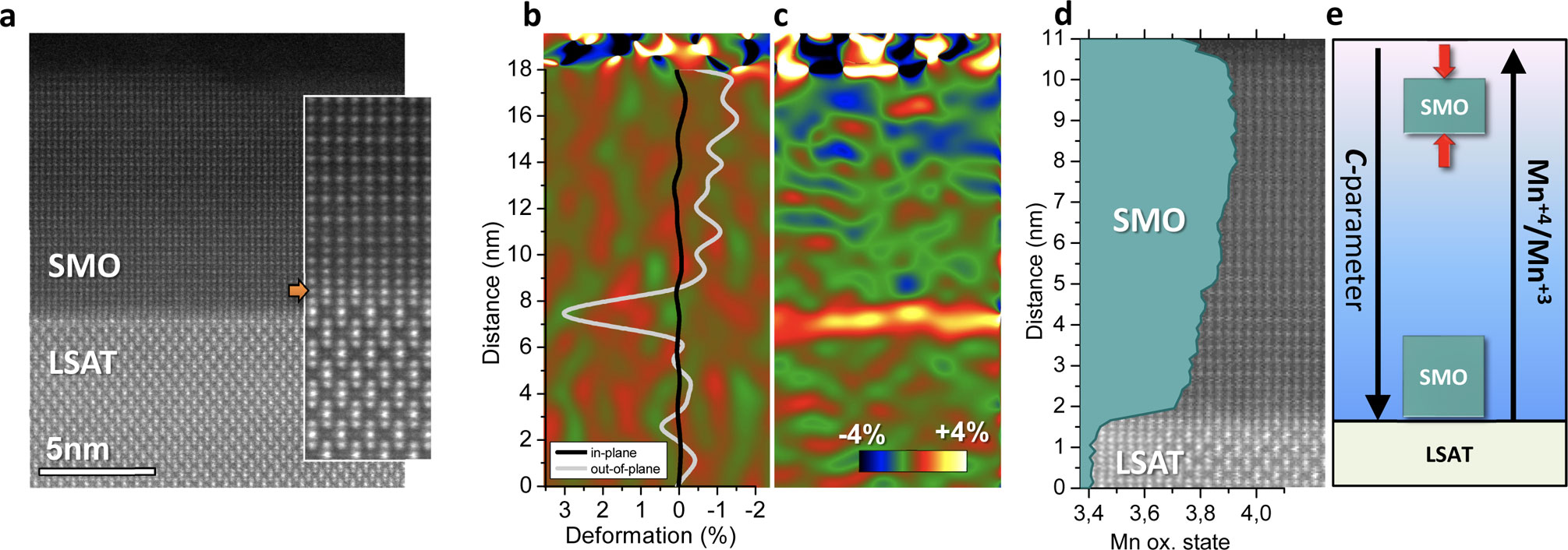
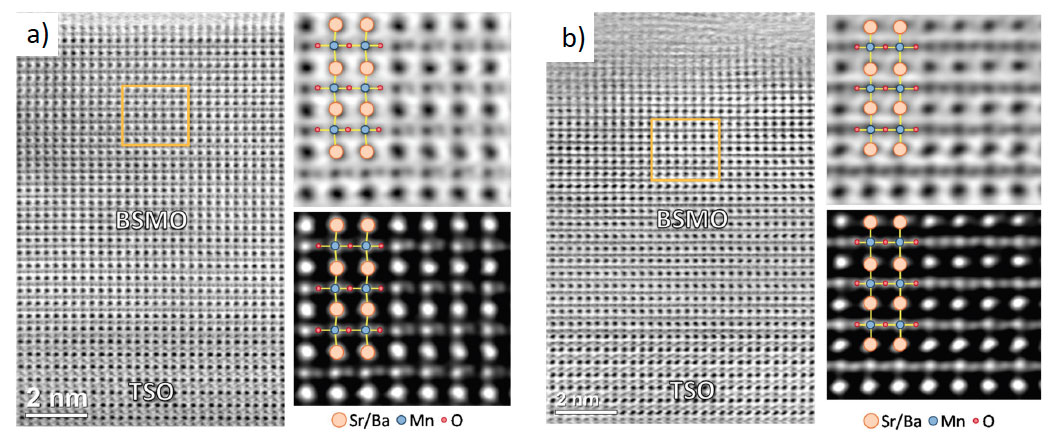
HIGHLIGHTS
Artificial chemical and magnetic structure at the domain walls of an epitaxial oxide. S. Farokhipoor, C. Magén, S. Venkatesan, J. Íñiguez, C.J.M. Daumont, D. Rubi, E. Snoeck, M. Mostovoy, C. de Graaf, A. Müller, M. Döblinger, C. Scheu and B. Noheda. Nature 515, 379–383 (2014).
Polar-Graded Multiferroic SrMnO3 Thin Films.
Roger Guzmán, Laura Maurel, Eric Langenberg, Andrew R. Lupini, Pedro A. Algarabel, José A. Pardo, and Ceśar Mageń.
Nano Letters 16, 2221-2227 (2016).
Controlling the Electrical and Magnetoelectric Properties of Epitaxially Strained
Sr1−xBaxMnO3 Thin Films.
Eric Langenberg, Laura Maurel, Noelia Marcano, Roger Guzmán, Pavel Štrichovanec, Thomas
Prokscha, César Magén, Pedro A. Algarabel and José A. Pardo.
Advanced Materials Interfaces 4, 1601040 (2017).
Laboratorio de Microscopías Avanzadas
We are a unique initiative at national and international levels. We provide the scientific and industrial community with the most advanced infrastructures in Nanofabrication, Local Probe and Electron Microscopies for the observation, characterization, nanopatterning and handling of materials at atomic and molecular scale.
Contact information
Campus Río Ebro, Edificio Edificio I+D+i
Direct Links
© 2021 LMA | Website developed by o10media | Política de privacidad | Aviso legal | Condiciones de uso | Política de Cookies |